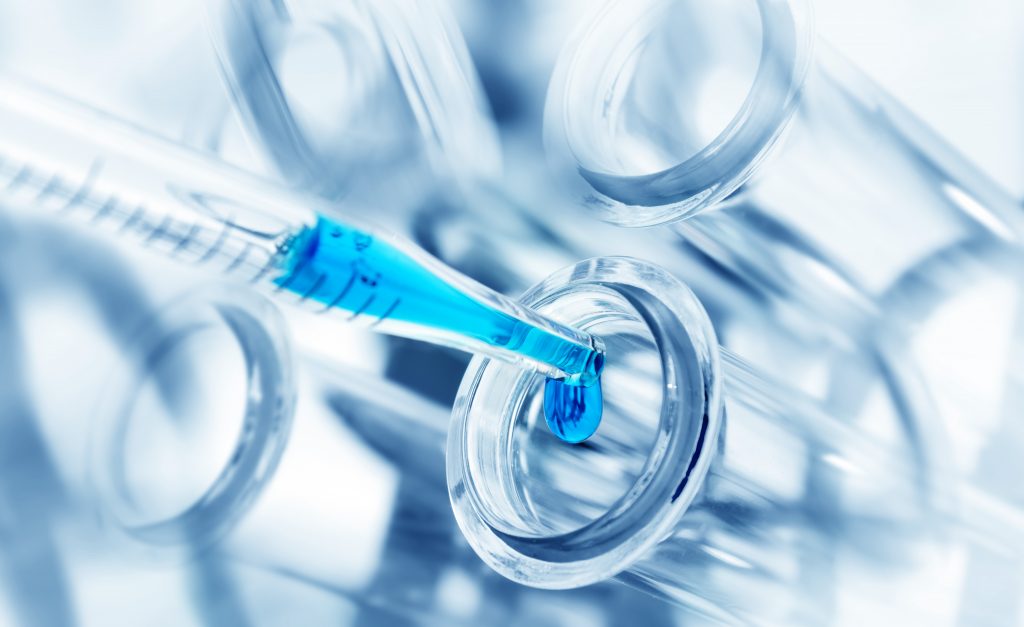
Reduced Nicotinamide Mononucleotide (NMNH) Potently Enhances NAD+, Causes Reductive Stress, and Suppresses Glycolysis and Cell Growth
Yan Liu 1,2,4, Chengting Luo 1,2,4, Ting Li 2,4, Wenhao Zhang 1, Zhaoyun Zong 2, Xiaohui Liu 2,3*, Haiteng Deng 2, 3, 5,*
1Tsinghua University-Peking University Joint Center for Life Sciences, Tsinghua University, Beijing, 100084, China
2MOE Key Laboratory of Bioinformatics, Centre for Synthetic and Systems Biology, School of Life Sciences, Tsinghua University, Beijing, 100084, China
3National Center for Protein Science, Tsinghua University, Beijing, 100084, China
4These authors contributed equally
ABSTRACT
In the present study, we developed a chemical method to produce dihydro nicotinamide mononucleotide (NMNH), which is the reduced-form of nicotinamide mononucleotide (NMN). We demonstrated that NMNH was a better NAD+ enhancer compared to NMN both in vitro and in vivo mediated by NMNAT. More importantly, NMNH significantly increased the reduced NAD (NADH) levels in cells and in mouse liver, resulting in cellular reductive stress and elevated reactive oxygen species. NMNH also induced cell cycle arrest and suppressed cell growth. Metabolomic analysis revealed that NMNH inhibited glycolysis and TCA cycle. However, NMNH treatment did not cause observable difference in mice. Taken together, our work demonstrates that NMNH is a potent NAD+ enhancer, and induces reductive stress to suppress cell growth.
RESULTS
NMNH increased cellular NAD+ levels
We investigated enzymatic and chemical synthesis methods for preparing NMNH and found that NMNH generated from chemical synthesis had a high yield and high purity. The procedure for NMNH synthesis is displayed in Figure 1A. NMNH was produced by reduction of NMN using thiourea dioxide (TDO). We chose TDO as the reducing reagent based on the facts that TDO is stable in common laboratory storage conditions, and it is amenable to solution preparation. TDO possesses high reduction potential in aqueous solutions and is also suitable for scalable applications. Briefly, 340 mg NMN and 125 mg TDO were dissolved in 1 mL of 10% ammonia solution. The
reaction mix was incubated at 40℃ for 1 hour and the product was then purified by HPLC using an amide column followed by vacuum drying. Purified NMNH was in orange color and had a UV absorption centered at 340 nm (Figure S1A). The reaction product was characterized by high resolution mass spectrometry. The mass to charge ratio (m/z) of the final product was determined to be 335.0648 in negative ion mode, which matches the theoretical molecular weight of NMNH (Figure S1B) with an elemental composition of C11H17N2O8P1. These results suggest that the reduction product is NMNH.
To further verify the identity of the reaction product, we generated NMNH from NADH using NADH pyrophosphatase (NudC), which decomposes NADH into NMNH and AMP (Frick and Bessman, 1995). The NADH decomposition reaction is shown in Figure S1C. The NudC-generated product was analyzed using high resolution mass spectrometry and the major peak was observed with mass to charge ratio (m/z) 335.0633 in negative ion mode (Figure S1D), precisely matching the mass of the reduction-generated NMNH. We compared the MS/MS spectra of enzymatically- and chemically-generated NMNH and found that they shared an identical fragmentation pattern (Figure S1E). Therefore, we confirmed that reduction of NMN by TDO produces NMNH. These results show that this reduction method is robust and effective. We examined the stability of NMNH and NMN and found that NMNH was stable under alkaline pH and low temperature conditions (Figure S1F). Unlike NMN, NMNH was unstable at neutral pH in solution (Figure S1F). The half-life of NMNH at pH 7.0 and at
room temperature was about 2.4 days while the half-life of NRH was 3 h at pH 6.0 and
at room temperature (Yang et al., 2019). We also found that NMNH was less stable than NMN in cell medium (Figure S1G). Cellular uptake of NMNH and NMN was quantitated by measuring their contents in cells and in medium over time using mass spectrometry method, as shown in Figure S1H.
We next examined the effects of NMNH on enhancing cellular NAD+ levels as compared to NMN. NMNH (100 μM) treatment increased the level of cellular NAD+ by 5-7 folds in HepG2 cells whereas 100 μM NMN only slightly increased the level of NAD+, as determined by mass spectrometry (Figure 1B and Figure S1I). These results indicate that, compared with NMN, NMNH is a more efficient NAD+ enhancer. In addition to HepG2 cells, we also confirmed that NMNH enhanced the NAD+ in ES-2 cells and 3T3-L1 cells, which are human ovary derived cells and mouse embryo fibroblast derived cells, respectively (Figure 1C and 1D). We found that NMNH increased cellular NAD+ levels in time- and concentration-dependent manner in 786-O cells (Figure S1J and S1K). Sirtuins are key NAD+-dependent deacetylases that play important roles in regulating biological functions, such as stress-resistance and DNA damage and generates NAM as one of the end products (Imai and Guarente, 2014). We examined cellular lysine-acetylation and found that both NMN and NMNH promoted cellular deacetylation activity with NMNH being more efficient than NMN (Figure S1L). We also found that NMNH increased cellular NAM concentration (Figure 1E).
We further examined the effects of NMNH on NAD+ levels using a mouse model.
We treated C57BL/6J male mice with 340 mg/kg NMN or NMNH via intraperitoneal
injection and measured liver NAD+ concentrations by mass spectrometry. We found that the liver NAD+ level was 4-fold higher in NMNH-treated mice than that in PBS- treated mice (Figure 1F). Additionally, the liver NAD+ level in NMNH-treated mice was 1.5-fold higher than that in NMN-treated mice. These results demonstrate that NMNH is a more potent in vivo NAD+ enhancer than NMN. NMNH also significantly increased levels of NAM in mouse liver (Figure 1G). We also examined the NAD+ enhancing effect of NMNH by oral delivery into mice and found that liver NAD+ level was increased by nearly 4 folds (Figure S1M).
6
A B
O
O P O OH
O
NH2
N
OH
O P O OH
O 5
NH2 4
3
O
OH OH
TDO, 1.2 equiv.
NH3ꞏH2O, 10%
40℃, 1h
2
1
OH OH 0
Nicotinamide Mononucleotide NMN
Reduced Nicotinamide Mononucleotide NMNH
C D E
4 7
5 6
4 3 5
3 2 4
3
2
1 2
1 1
0 0 0
F G
6 2.0
1.5
4
1.0
2 0.5
0 0.0
Figure 1. NMNH is potent NAD+ booster which is more effective than NMN
(A)NMNH synthesis procedure. NMNH was generated by reducing NMN with TDO under alkaline environment at 40 ℃ for 1 h.
(B)NMNH had better NAD+ enhancing effect than NMN in HepG2 cell. Cellular NAD+ concentration was determined after 100 μM NMN or NMNH treatment for 12 h using TSQ Quantiva mass spectrometer. Data are shown as mean ± SD (n = 4).
****p < 0.0001. Significance is obtained compared to the untreated group unless otherwise specified. (C)NMNH increased cellular total NAD(H) concentration in ES-2 cell. Cellular total NAD(H) concentration was determined after 100 μM NMN or NMNH treatment for 3 h using NAD+/NADH detecting kit (Beyotime, China). Data are shown as mean ± SD (n = 3). *p < 0.05, ***p < 0.001. Significance is obtained compared to the untreated group unless otherwise specified. (D)NMNH increased cellular total NAD(H) concentration in 3T3-L1 cell. Cellular total NAD(H) concentration was determined after 100 μM NMN or NMNH treatment for 3 h using NAD+/NADH detecting kit (Beyotime, China). Data are shown as mean ± SD (n = 3). *p < 0.05, ****p < 0.0001. Significance is obtained compared to the untreated group unless otherwise specified. (E)NMNH increased cellular NAM concentration in HepG2 cell. Cellular NAM concentration was determined after 100 μM NMN or NMNH treatment for 12 h using Q Exactive mass spectrometer. Data are shown as mean ± SD (n = 4). **p < 0.01, ****p < 0.0001. Significance is obtained compared to the untreated group unless otherwise specified. (F)NMNH had better in vivo NAD+ enhancing effect than NMN. C57BL/6J male mice were treated with 340 mg/kg NMN or NMNH for 6 h by intraperitoneal injection. Liver NAD+ concentration was determined using TSQ Quantiva mass spectrometer. Data are shown as mean ± SD (n = 5). *p < 0.05, **p < 0.01, ****p < 0.0001. Significance is obtained compared to the untreated group unless otherwise specified. (G)NMNH increased liver NAM concentration. C57BL/6J male mice were treated with 340 mg/kg NMN or NMNH for 6 h by intraperitoneal injection. Liver NAM concentration was determined using TSQ Quantiva mass spectrometer. Data are shown as mean ± SD (n = 5). *p < 0.05. Significance is obtained compared to the untreated group unless otherwise specified. A B C 3.5 3.0 2.5 2.0 1.5 1.0 0.5 0.0 D E 70 **** 60 50 40 30 20 10 0 Untreated 1 mM NMNH 1 mM NMN **** 2.5 2.0 1.5 1.0 0.5 0.0 0 24 48 72 96 120 G1 S G2/M Culture Time (hours) F 100 75 50 25 0 786-O HK-2 Untreated 10 M NMNH 50 M NMNH 100 M NMNH 250 M NMNH 500 M NMNH Figure 2. NMNH caused reductive stress and repressed cell growth (A)NMNH increased cellular NADH concentration in HepG2 cell. Cellular NADH concentration was determined after 100 μM NMN or NMNH treatment for 12 h using TSQ Quantiva mass spectrometer. Data are shown as mean ± SD (n = 4). *p < 0.05, ***p < 0.001. Significance is obtained compared to the untreated group unless otherwise specified. (B)NMNH increased cellular ROS level in HepG2 cell. Cellular ROS were determined after 500 μM NMNH treatment using CellROX® Deep Red Reagents (Invitrogen, NY). Data are shown as mean ± SD (n = 3). *p < 0.05, ***p < 0.001. Significance is obtained compared to the untreated group unless otherwise specified. (C)High concentration NMNH inhibited the growth of HepG2. Cell growth rates were determined after NMNH treatment for 72 h using CCK-8 (Dojindo, Kumamoto, Japan). Data are shown as mean ± SD (n = 3). ****p < 0.0001. Significance is obtained compared to the untreated group unless otherwise specified. (D)Percentages of G1phase, S phase, and G2/M phase subpopulations in differently treated HepG2 cells by cell cycle analysis. Data are shown as mean ± SD (n = 5). ****p < 0.0001. Significance is obtained compared to the untreated group unless otherwise specified. (E)Growth curve of 786-O under 500 μM NMNH treatment. Cell growth rate were determined under 500 μM NMNH treatment using CCK-8 (Dojindo, Kumamoto, Japan). Data are shown as mean ± SD (n = 3). ****p < 0.0001. Significance is obtained compared to the untreated group unless otherwise specified. (F)786-O is more sensitive to NMNH compared to HK-2. Cell growth rates were determined after NMNH treatment for 72 h using CCK-8 (Dojindo, Kumamoto, Japan). Data are shown as mean ± SD (n = 3). **p < 0.01, ***p < 0.001, ****p < 0.0001. Significance is obtained compared to the untreated group unless otherwise specified. A DHAP Glucose Glucose-6-P Fructose-6-P Fructose-1,6-BP GAP 1,3-BPG Glucose 80 60 40 20 0 M+0 M+6 Glucose-6-P **** 80 **** 60 40 20 0 M+0 M+6 Fructose-6-P 80 p=0.052 p=0.052 60 40 20 0 M+0 M+6 3PG 2PG PEP B Oxaloacetate Oxaloacetate Pyruvate Pyruvate CO2 Acetyl-CoA Citrate 100 80 60 40 20 **** -ketoglutarate **** *** *** **** * Malate Fumarate TCA cycle Succinate Isocitrate CO2 α-Ketoglutarate CO2 Succiny-CoA 0 M+0 M+1 M+2 M+3 M+4 M+5 Figure 3. NMNH repressed glycolysis and TCA cycle (A)Left, schematic of glycolysis. Red solid circle represents 13C. Right, percentages of different isotope-encoded glycolysis metabolites. M+n represents a metabolite contains n 13C atoms. Cellular metabolite concentrations were determined after 1 mM NMN or NMNH treatment in media supplied with U-13C6 glucose for 6 h using TSQ Quantiva mass spectrometer. Data are shown as mean ± SD (n = 4). *p < 0.05, ***p < 0.001, ****p < 0.0001. Glucose-6-P, glucose-6-phosphate. Fructose-6- P, fructose-6-phosphate. Fructose-1,6-BP, fructose-1,6-bisphosphate. GAP, glyceraldehyde-3-phosphate. DHAP, dihydroxyacetone phosphate. 1,3-BPG, 1,3- bisphosphoglycerate. 3PG / 2PG, 3-phosphoglycerate / 2-phosphoglycerate. PEP, phosphoenolpyruvate. Significance is obtained compared to the untreated group unless otherwise specified. (B)Left, schematic of TCA cycle. Red solid circle represents 13C. Right, percentages of different isotope-encoded TCA cycle metabolites. M+n represents a metabolite contains n 13C atoms. Cellular metabolite concentrations were determined after 1 mM NMN or NMNH treatment in media supplied with U-13C6 glucose for 6 h using TSQ Quantiva mass spectrometer. Data are shown as mean ± SD (n = 4). *p < 0.05, **p < 0.01, ***p < 0.001, ****p < 0.0001. Significance is obtained compared to the untreated group unless otherwise specified. A O H2N O H2N OH OH OH OH O O O O O O NAD+ M+0 O O O O NAD+ OH OH OH OH NAD+ 80 60 40 20 0 C 80 60 40 20 0 NADH O H2N M+5-N D NN OH OH O O O O O + OH OH O HO + + HO O H2N NAD M+5-A OH OH ATP ATP NMNAT NMNAT OH OH O O O O O O H2N OOH NH2 NN O H2N OH OH NH2 NN N OP P OO O O N N N O P P OO O O N N OH OH NAD+ OH OH O O OH OH OH OH O O OH OH OH OH M+10 NAD+ NADH Figure 4. NMNH-induced NAD+ synthesis pathway Deconstructing (A)NAD+ label patterns. Red dots and blue dots represent 13C atoms. (B)Percentages of different isotope-encoded NAD+. M+n represents NAD+ contains n 13C atoms. Cellular NAD+ concentrations were determined after 1 mM NMN or NMNH treatment in media supplied with U-13C6 glucose for 6 h using TSQ Quantiva mass spectrometer. Data are shown as mean ± SD (n = 4). **p < 0.01, ****p < 0.0001. Significance is obtained compared to the untreated group unless otherwise specified. (C)Percentages of different isotope-encoded NADH. M+n represents NADH contains n 13C atoms. Cellular NADH concentrations were determined after 1 mM NMN or NMNH treatment in media supplied with U-13C6 glucose for 6 h using TSQ Quantiva mass spectrometer. Data are shown as mean ± SD (n = 4). *p < 0.05, ****p < 0.0001. Significance is obtained compared to the untreated group unless otherwise specified. (D)Proposed metabolic pathway through which NMNH is synthesized to NAD+